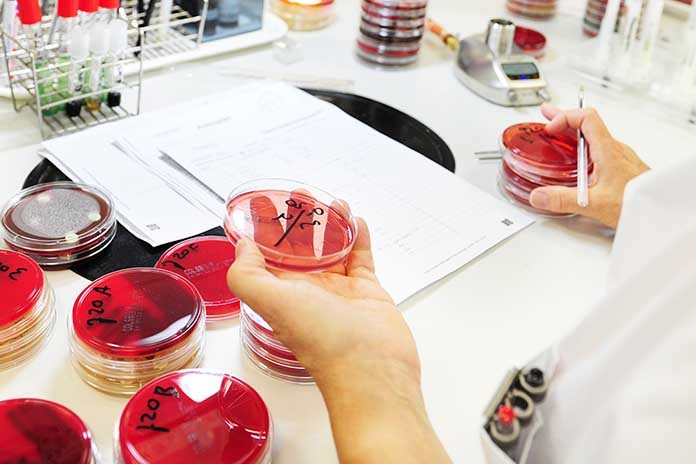
Detection of infectious diseases can generally be broken down into laboratory detection of the pathogen or detection of the immune response to the pathogen. Despite which direction is chosen, there are multiple methods to use for detection. This can create challenges, as there is no standardization of methods among diagnostic labs (except for NAHLN testing). Results usually cannot be compared between labs because of the different methods, pathogen strains, etc. used. This is especially true for serological testing. Additionally, with the introduction of molecular methods, which are highly sensitive, we begin questioning the significance of a positive result.
Cultivation
The focus of this presentation is pathogen detection. As mentioned, there are several methods that may be used to do this. The first method (and historically considered the gold standard method) is cultivation, which is culture for bacteria/fungi and isolation for viruses. The important thing to consider for this method is the viability of the organism. If the clinical sample has not been handled properly, we will not detect the organism with this method. Additionally, if trying to culture a bacterium, it is important to let the lab know which organisms you are interested in detecting. We use a special media for organisms like Mycoplasma. Additionally, proper sample collection is important for culture. Detection of environmental contaminants/normal flora (microbiota) will not help you make the diagnosis. The beauty of culture/isolation is that these methods are rather non-specific. You just need the right media (bacteria/fungi) or eggs/cell culture (virus) to detect organisms. However, not all organisms can be grown in vitro or are difficult to cultivate, so a different method for detection would then be better. Also, virus isolation can take weeks to get a result and just like with bacteria, ID of the organism following cultivation requires additional steps, such as use of specific antibodies that bind to the virus or electron microscopy to visualize the virus.
Electron microscopy (EM)
Another way to detect viruses is by electron microscopy. The availability of this method is declining. Many labs no longer offer EM because as the equipment has aged, labs do not have the funds to replace the machines and maintenance of the old equipment is too expensive. Some labs (such as the ADDL) now depend on university core facilities that have the electron microscope to run their diagnostic samples. This hampers turn-around time and can result in increased cost to the client to off-set costs of running the samples in the core facility. Unlike with cultivation, organisms do not need to remain viable for detection by EM. Like cultivation, the method is non-specific (can detect many different viruses). EM is commonly used to detect viruses associated with enteric disease. EM is beneficial in this situation because these viruses are generally shed in high amounts (106 viral particles needed for detection by EM) and also many of these viruses are difficult to cultivate in vitro. However, molecular methods have/are replacing EM for detection of viruses.
Antigen detection
Antigen detection is detection of the pathogen by its antigens (usually protein). This method is generally specific because of the antibody used, particularly if a monoclonal antibody is used (binds to a specific epitope). If polyclonal antibodies are used, the antibodies may cross-react with unintended pathogens. However, the benefit of using polyclonal antibodies is there is less worry about missing the pathogen if, for example, there has been a change in the epitope that would bind a monoclonal antibody.
The pathogen needs not be viable for this method of detection. Methods for antigen detection include (but are not limited to) fluorescent antibody tests, immunohistochemistry, and ELISA. In general, ELISAs are more commonly used for antibody detection rather than antigen detection, but one well know antigen ELISA is the BVDV ACE (antigen capture ELISA) for detection of PI animals. The pros and cons of each antigen detection method will not be addressed.
Genetic detection
Polymerase Chain Reaction (PCR) involves repeated cycles of amplification of a targeted region of a pathogen’s genome. Primers (small pieces of complementary DNA) are designed to bind to the pathogen of interest (and theoretically nothing else). Therefore, this method will only find what you are specifically looking for in the sample (specific method).
These primers are also necessary for the reaction to occur because the DNA polymerase needs the primers to “prime” or start the DNA synthesis. The DNA polymerase will only produce complementary DNA strands, so if a pathogen is RNA (ie RNA virus), the genetic material must first be converted to DNA before PCR can take place (this is called RT (reverse transcription)-PCR). We include both a forward and reverse primer in the mix (DNA is double stranded- 1 primer per strand). Free nucleotides (A,T,C,G) are included in the mix and are added by the polymerase to the primer ends for a growing strand of DNA that is complementary to the pathogen DNA. The reaction cycles include separation of the double stranded DNA in the sample (denaturation), binding of the primers to the complementary DNA strands of the pathogen if present in the sample (annealing), and extension of the primers by the DNA polymerase (elongation). Each of these steps requires a different temperature, which is why the reaction is performed in the thermocycler (cycles between different temps). Amplification of a portion of the pathogen genome with each PCR cycle (amount of PCR product doubles with each cycle) results in the sensitivity of the method. Because of the exquisite sensitivity of the method, it is important for the lab to take the appropriate precautions and use adequate controls to prevent/detect contamination between samples to avoid reporting false-positive results.
Conventional PCR
Conventional PCR has many names, including traditional PCR, gel-based PCR, and end-point PCR. Basically, this method is used to visualize the PCR product at the end of the run. With all the amplification that is done in the reaction, there is a great deal of product produced. So much in fact that it is visible on a gel. Based on where the primers are expected to bind the pathogen genome tells us how big of a product we expect to produce. We include a DNA ladder on the gel to determine band size of the PCR product. Occasionally something unexpected happens, we amplify a non-specific product (not our intended pathogen) that is of the same size as our expected product. Therefore, ideally, we will use an additional method (such as sequencing) to confirm that our product is actually what we expect it to be. Sequencing will be further discussed below.
Real-time PCR
This is a variation on conventional PCR. Instead of using a gel to detect the PCR product at the end of the reaction, we incorporate a fluorescent probe into the reaction to detect the product. This probe, when intact, does not produce fluorescence. The intact probe has a fluorescent tag on one end and a quencher on the other end. The quencher quenches the fluorescence. Like the primers, the probe is DNA that is complementary to the pathogen DNA. It is designed to bind to the pathogen DNA between the primers and it binds first. The addition of a probe to the PCR reaction increases test specificity because now there are 3 pieces of complementary DNA that have to bind the pathogen rather than just the 2 primers. When the polymerase extends from the primers, it chops up the probe as it reads through the sequence. This results in fluorescence (fluorescent tag is now separated from the quencher), which is detected by the system. Therefore, we can detect amplification in “real-time,” as the PCR product is being made. There is always some amount of probe that is not intact, and this will create some background fluorescence. The system subtracts this out by setting a baseline, and a threshold is determined for each sample (point at which the sample is considered positive). If there is lots of pathogen in the sample, there will be lots of probe binding with break down by the polymerase. This will results in an increase in fluorescence faster than if there is little pathogen in the sample, which will take longer for the fluorescence produced to meet the threshold. Obviously, if the pathogen is not in the sample, the probe will not be broken down, and we will only see background fluorescence in the reaction. The PCR cycle at which the fluorescence passes the threshold is called the threshold cycle (Ct). So, a lower Ct value represents more pathogen in the sample. Ct values over 35 are generally considered questionable/suspect (and may not be reproducible because this represents very small amount of pathogen DNA in the sample). The importance put on a Ct value this high depends on what you are trying to determine with the test. For example, if the test is being used for surveillance in a clinically healthy group of animals, a Ct value ≥ 35 could be a significant finding. One needs to consider the result in light of clinical signs and stage of infection. Detection of a pathogen does not necessarily mean the pathogen is the cause of the disease. It might be a contributor or an incidental finding. Although, in general, a low Ct value is a significant finding. Real-time PCR can be used to determine copy number of a pathogen in a sample, but a standard curve is needed for this. This is not generally done in animal diagnostics.
Sequencing
There are two main methods for sequencing. These are Sanger sequencing and Next Generation Sequencing. Sanger sequencing has been around much longer and is used to sequence a PCR product-generally obtained by conventional PCR. This method also requires primers and is very useful if you know what you are looking for in the sample. It is commonly used to sequence genes/portions of pathogens, and this is the most common sequencing method currently used in veterinary diagnostics.
Sequencing can be used to speciate bacteria that are difficult to speciate with biochemicals (or Maldi Tof) alone. This method can also be used to differentiate infection from modified-live vaccine shedding or to differentiate disease caused by a vaccine versus a wild-type strain. Once we obtain the sequence, we determine what the sequence is by using a government database of known sequences (BLAST-https://blast.ncbi.nlm.nih.gov/Blast.cgi) to compare our sequence. Sequencing is also useful for molecular epidemiology for outbreak tracing. To do this, we also use phylogenetic analysis to compare between multiple sequences.
Phylogenetic analysis
Phylogenetic trees are used to evaluate how sequences are related (evolutionary relationships among a set of organisms). As sequencing has become more common in diagnostic labs, the need to compare sequences and to prepare and interpret phylogenetic trees has grown. It is important to understand that there is no right/correct method or tree. The methods used only provide inferences. Some methods are considered more accurate than others, but several different methods are scientifically acceptable.
The key is producing a tree with clades that are reproducible. A clade is a group of organisms that includes a single ancestor and all of its descendants (for our purposes, closely related sequences). Reproducibility of the tree clades can be determined by using the bootstrap method, which estimates the probability of whether a split in the tree is correct (the probability that the members of a given clade are always members of that clade). This percentage (boostrap value) appears adjacent to the node and provides the support to the branch connecting to that node. The higher the number at the node, which represents the common ancestor of the clade, the better (want it to be ≥70% – means it showed up in 70+/100 iterations of the tree). We want a tree that is consistent with the data and most closely approximates what happened in the past (if we are concerned about evolution of the virus/bacteria). Another thing to evaluate on a phylogenetic tree is the branch length. Branch length represents the amount of change between an ancestor and its descendants (or for our purposes, the amount of nucleotide change). The longer the branch length, the greater the number of nucleotide changes. A key is provided with the tree-number of nucleotide changes per site. The majority of these nucleotide changes will be silent (no change to the amino acids/protein). So, branch length does not represent antigenic changes, just nucleotide changes. Therefore, just because there are nucleotide changes resulting in a new clade of virus does not mean current vaccines are not protective. Antigenic changes must be evaluated by a different means.
Next Generation Sequencing (NGS)
This is the newest molecular technique to revolutionize medicine and diagnostics. This sequencing method varies from Sanger in that it does not require primers for the sequencing reaction and DNA strands are sequenced in parallel, not 1 single strand per reaction. So, theoretically, one could sequence all the nucleic acid in a given sample (this is called metagenomics, shot-gun sequencing, or deep sequencing). NGS is more widely used for sequencing genomes of bacteria or viruses that have been isolated from clinical samples or for sequencing human/animal/plant genomes (whole genome sequencing-WGS) than for metagenomics. WGS is used by the FDA and state health departments to evaluate the genetic epidemiology of foodborne pathogen outbreaks. Evaluation of human genomes is leading to “precision medicine” that is tailored to an individual’s genetic composition. In the case of pathogen detection, metagenomics is very useful for detecting new organisms. The complication is determining if these “new” organisms are pathogens and responsible for the observed clinical disease. Additionally, because there is no need for primers for NGS, there is no need to request specific tests for all the organisms on your differential list. However, to do a true metagenomics run costs several hundred, up to $1000 dollars. The amount of pathogen DNA/RNA in a sample, particularly a tissue sample with lots of animal cells, is very small compared to the amount of animal nucleic acid.
Therefore, millions of sequencing reads are needed to find the pathogen. An approach to reduce costs with NGS is to incorporate PCR primers into the workflow. This limits the method to detecting just those pathogens for which primers are included, but it significantly reduces the number of reads required to detect the pathogen, and therefore, reduces the cost. This approach also still allows detection of a large number of pathogens in a single sample, making it a highly useful technique for syndromic testing. Multiplex PCR assays are commonly used to detect multiple pathogens in a sample, but this method is limited to few targets. Also, unlike with NGS, no sequence data is obtained with PCR alone. That must be done through a sequencing reaction. NGS is useful because areas that we would have normally targeted with Sanger sequencing can now be incorporated into this test. So, it is one test and done-detect and characterize in a single test. Therefore, targeted NGS is like multiplex PCR on steroids with sequencing. Targeted NGS can be run in as few as 2 days once received by the lab. While I worked at UGA, we developed a panel for detection of pathogens in cows and small ruminants. We hope to incorporate this type of testing into the ADDL and develop additional panels.
From the 2019 Proceedings of the Midwest Poultry Federation Convention